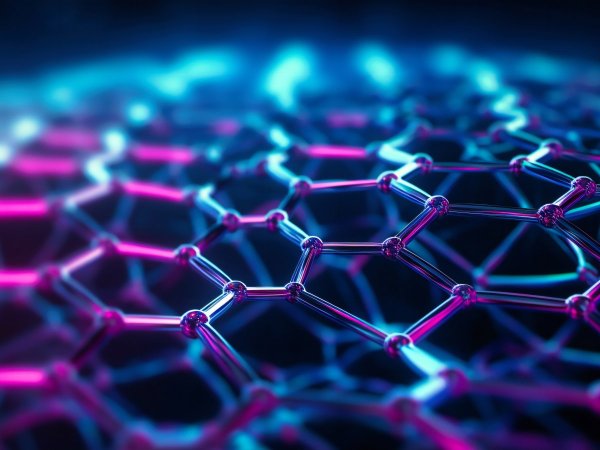
All materials are made up of atoms. These atoms have a nucleus that contains protons and neutrons while electrons orbit the nucleus. Most materials and their electrons obey the laws of classical physics. For example, we can understand if a material will conduct electricity well by considering how many electrons it has and how easy it is for those individual electrons to move. However, there is another class of materials in which classical physics is not sufficient to explain their properties. These are called quantum materials. Their properties can only be explained by using quantum mechanics, which is a branch of physics that deals with the behavior of things at the atomic level. To understand quantum materials, it requires considering the behavior of many electrons simultaneously by using quantum mechanics.
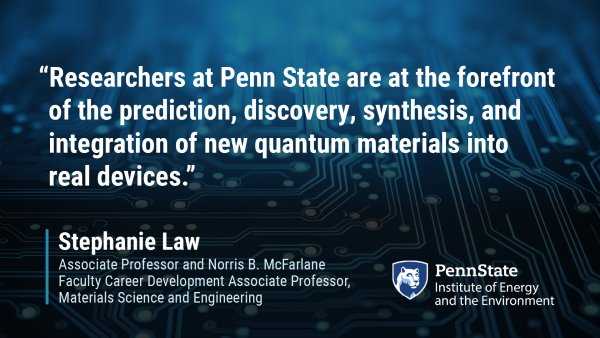
Quantum materials include superconductors, where pairs of electrons move together; magnets, where the spin of one electron influences the spin of its neighbor; topological materials, where the electrons on the surfaces of the materials have exotic properties; and quantum-confined materials, where the electron properties are determined by the size of the material. Although these materials may seem complicated and mysterious, their unusual properties could be harnessed to help solve challenges with energy transmission and data storage.
Computer chips are made up of billions of transistors, each of which can be placed in a 0 or 1 state, the binary code used in computing systems. Computing operations are performed by flipping the state of the transistor. State-of-the-art transistors have dimensions of only a few nanometers, which corresponds to only a few layers of atoms. Shrinking transistors further can put more computing power on the same chip, but we are reaching the limits of what can be achieved using traditional materials. One solution to this problem is two-dimensional (2D) materials. 2D materials typically comprise one or more layers of atoms that are strongly bonded together in two directions, making a sheet of material. A relatively popular example of this is graphene, a material that is one million times thinner than paper and potentially the strongest material in the world. These materials can have outstanding properties and would allow device designers to make transistors much smaller than can be done with traditional materials.
In addition to transistors, computer chips also contain interconnects. These are tiny wires that allow information to be routed on the chip. Most state-of-the-art chips use copper interconnects. However, as current flows through copper interconnects, the electrons lose some of their energy due to resistance caused by electrons bouncing off defects in the copper as it travels through a circuit. A significant fraction of the energy used by computer chips is lost to heat in the interconnects, which, in addition to wasting energy, also causes our devices to heat up. Quantum materials may offer a solution to this problem. Topological materials have a variety of unique properties, including surface states that are highly conducting in which the electrons “bounce around” less. They also have the potential for decreased resistance at decreased sizes. Though research into topological materials for interconnects is still in its infancy, they are extremely promising.
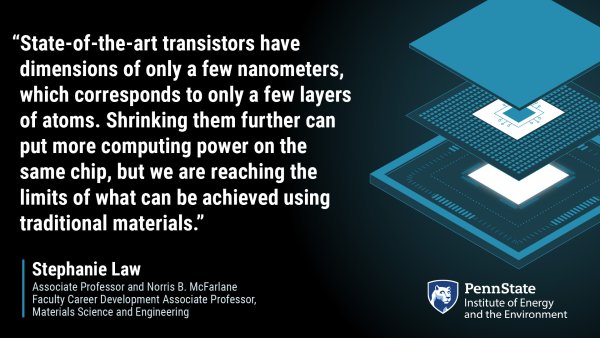
Another solution to the problem of energy use in computing is spintronics. Computers currently perform computations and store information by moving electrons around, which costs energy. In a spintronic system, the unit of information is not the electron charge, but the electron spin. “Spin” is a quantum mechanical property of the electron—the electron is not actually spinning like a top, but instead can be found in a “spin up” or a “spin down” state. Switching the direction of the electron spin from “up” to “down” can be much less energy intensive than moving the electron around. This could, in turn, allow us to perform computations and store information using much less energy. A variety of quantum materials are candidates for spintronics, including quantum dots, topological materials, and magnetic materials.
Finally, superconductors are a class of quantum materials that can transmit energy with no loss. A superconducting wire has zero resistance, so it can carry current without losing any energy to heat. This contrasts with wires made from copper which have a low but nonzero resistance and always lose energy to heat. Today, superconductors are primarily used to make magnets for magnetic resonance imaging machines, though there have been small-scale demonstrations of wires made from superconductors for electrical power transmission and magnetic levitation trains. The main roadblock to additional adoption of superconductors for lossless power transmission is that existing superconductors do not work at room temperature. Current research is focused on predicting and synthesizing materials that are room-temperature superconductors.
We can clearly see that quantum materials have many applications in the field of energy technologies. However, we are currently limited by the availability of materials that meet our needs. Researchers at Penn State are at the forefront of the prediction, discovery, synthesis, and integration of new quantum materials into real devices.
Stephanie Law is the Norris B. McFarlane Faculty Career Development Associate Professor in the Department of Materials Science and Engineering and an IEE faculty member. Her research group synthesizes thin films and heterostructures using molecular beam epitaxy for energy applications. In particular, their efforts center around photonic materials and metamaterials spanning the electromagnetic spectrum from the visible through the terahertz range. These materials have applications in solar cells, efficient lighting, waste heat recovery, photocatalysis, and more.